
|
The Search for "Heavy" Elements

When a nucleus captures a neutron, it often tries to correct for its neutron excess by beta decay, turning a neutron into a proton and thus creating an atom with atomic number Z increased by one unit. This commonly observed phenomenon suggests a way to create new elements of increased atomic number and thus to create ever more massive elements that are not found on Earth. Most of these elements are radioactive, with very short half-lives. However, theories of nuclear structure predict that at a certain atomic number, which is currently beyond present experimental limits, new long-lived nuclei can be created.
The most massive naturally occurring element on Earth is uranium (U), with a nucleus of 92 protons. In 1934, scientists started the search for more massive elements with 93 or more protons. They succeeded in 1940 when neptunium (Np, Z = 93) was synthesized at the University of California, Berkeley. Edwin McMillan and Philip Abelson observed Np while studying fission products produced in the bombardment of 238U with thermal neutrons. They found a radioactive reaction product that was not a fission product. This product was formed by the capture of a neutron to produce 239U, which subsequently b- decayed to 239Np via the reaction
239U + 1n Æ 239U (23.5 min) Æ 239Np (2.36 d),
where the half life of the nucleus is indicated in the parenthesis. McMillan and Abelson chemically separated this new element, Np, from the interfering fission products and chemically identified it as neptunium. Since this breakthrough discovery, scientists from all over the world have been trying to discover ever more massive artificially produced elements.
Plutonium (Pu, Z = 94) was discovered in 1941 by bombarding a uranium target with deuterons (a hydrogen nucleus with one proton and one neutron) in the 60-Inch Cyclotron at Berkeley. Glenn Seaborg, Arthur Wahl and Joseph Kennedy chemically separated neptunium from the target and detected alpha particles from the plutonium daughter nuclei, as:
238U + 2H Æ 2 1n + 238Np (2.1 d) Æ 238Pu (88 yr) .
They chemically identified the isotope 238Pu. Then, joined by Emilio Segre, they identified 239Pu and showed that it was fissionable with thermal neutrons.
Once 239Pu was discovered, there was the potential for using it as a new target to produce more massive elements. In 1944 a 239Pu target was bombarded with alpha particles at the 60-Inch Cyclotron to produce curium (Cm, Z = 96) through the following nuclear reaction
239Pu (24,110 yr) + 4He Æ 242Cm (162.8 d) + 1n.
After bombardment the material was sent to the Metallurgical Laboratory at The University of Chicago for chemical separation and identification of the new element. 242Cm decays to 238Pu by emitting alpha particles. The identification of curium was possible because the alpha decay of the daughter nucleus, 238Pu, was already known and could be used as a signature for the identification of the curium precursor.
The discovery of americium (Am, Z = 95) soon followed when a 239Pu target was bombarded with thermal neutrons in a nuclear reactor. Plutonium captured several neutrons and ultimately became americium (241Am):
239Pu (24,110 yr) + 1n Æ 240Pu (6,564 yr) + 1n Æ241Pu (14.4 yr),
241Pu (14.4 yr) Æ 241Am (432.7 yr) + 1n Æ 242Am (16.0 h) Æ 242Cm (162.8 d).
Americium was chemically separated from plutonium and further identified by observing its beta decay to the known 242Cm isotope.
Once americium and curium were discovered and isolated in macroscopic amounts, they were used as targets to produce more massive elements through particle bombardments. Berkelium (Bk, Z = 97) was produced by bombarding milligram quantities of americium with helium ions,
241Am (432.7 yr) + 4He Æ 243Bk (4.5 h) + 2 1n.
Rapid chemical techniques were developed in order to separate and identify this new short-lived element. Likewise, californium (Cf, Z = 98) was produced in a helium bombardment of a target made of microgram amounts of curium by
242Cm (162.8 d) + 4He Æ 245Cf (45 min) + 1n.
The identification of this element was accomplished with only the 5000 atoms produced in this experiment. The next two elements, einsteinium (Es, Z = 99) and fermium (Fm, Z = 100), were unexpectedly found in the debris from the "Mike" thermonuclear explosion that took place in the Pacific Ocean in 1952. Debris from the explosion was collected and analyzed at several laboratories, and the new elements were discovered in chemical separations of the material. Scientists explained the production of einsteinium and fermium through multiple neutron captures by the uranium used in the thermonuclear device followed by several successive beta decays, which ultimately resulted in atoms with atomic numbers 99 and 100.
The last three elements in the actinide series are mendelevium (Md, Z = 101), nobelium (No, Z = 102) and lawrencium (Lr, Z = 103). Mendelevium was truly a unique discovery because the new element was produced and identified virtually one atom at a time. Einsteinium was bombarded with helium ions to produce mendelevium through:
253Es (20.5 d) + 4He Æ 256Md (78.1 min) + 1n.
The production of mendelevium was estimated to be only a few atoms per experiment. The reaction products from the bombardment were collected on thin gold foils that were dissolved in an acid solution, and then chemically treated in order to separate and identify the Md atoms. This is commonly called the recoil method, and is used when small amounts of atoms are produced.
The discovery of nobelium was controversial. A team of scientists from several different laboratories claimed discovery in 1957. However, scientists from the United States and the Soviet Union could not confirm their findings. The original claim was proven to be false; the product that was thought to be nobelium was actually something completely different. Nobelium was finally produced and positively identified in 1958 through the following reaction:
246Cm (4730 yr) + 12C Æ 254No (55 s) + 4 1n.
The first identification of lawrencium was made at the Berkeley Labs Heavy Ion Linear Accelerator (HILAC) in 1961. Several targets of californium isotopes were bombarded with beams of boron. The reaction products were collected on a mylar tape and moved past a series of alpha detectors. The element lawrencium was identified on the basis of the known alpha decays of its descendant nuclei.
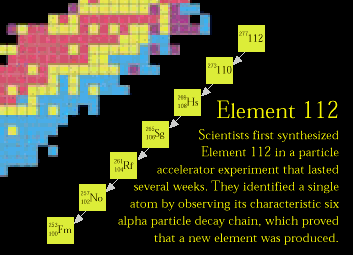
Once the actinide series was filled in the periodic table, work was started to produce the transactinide elements. The most recent version of the periodic table includes transactinide elements from rutherfordium (Rf, Z = 104) to element 112, which has not yet been named. As scientists tried to discover and identify the transactinide elements, they encountered several problems that made their task extremely difficult. The rates for producing these elements in accelerator bombardments were incredibly low. Consequently, only a few atoms could be produced over the course of the entire experiment. Sometimes these experiments went on continuously for several weeks. In addition, the half-lives of the transactinides are very short, making their identification a difficult challenge. Unlike neptunium and plutonium, these elements may undergo radioactive decay before being detected. As a result, chemical separations are generally very difficult to perform for identifying transactinides. In order to confirm that a new element has actually been made, members of its decay chain must be detected and traced back as a signature to the unknown element.
Elements 104 through 112 were discovered by observing the decay chain of their descendant nuclei. Only a few atoms of these new elements were produced in each experiment. The atoms were isolated from the target and beam material by using a particle separator which separates atoms based on their different masses. The atoms were then allowed to decay and the subsequent alpha particle decay products from the descendant nuclei were correlated to identify the unknown parent nucleus. In the discovery of element 112, the individual atoms were identified by the decay chains of parent-daughter-granddaughter nuclei (above). Different combinations of target and projectile were used in accelerators to produce these new elements.
Rutherfordium (104), dubnium (105), and seaborgium (106) were synthesized and identified at Berkeley. Bohrium (107), hassium (108), and meitnerium (109) were synthesized and identified in the early 1980s at the Gesellschaft für Schwerionenforschung (GSI) laboratory near Darmstadt, Germany. In the 1990s, unnamed element 110 was identified at the GSI, Berkeley, and Dubna laboratories, and elements 111 and 112 were found at the GSI laboratory. The names for these new elements are under discussion.
New experimental techniques and apparatus are being developed for scientists to extend the periodic table to even more massive elements. A more efficient particle separator, will use magnetic fields to separate atoms based on their mass and charge. This equipment will allow detection of nuclides with low production rates and extremely short half-lives. There are plans for using such a separator to produce elements 114 and 116. The present limits for discovering new elements are based on the low production rates and short half-lives. The hope is that new development in detection equipment will increase the sensitivity for detecting fewer atoms (or even a single atom) with very short half-lives. A widely used theory predicts the existence of elements up to Z = 125. In this model, more massive elements would be so unstable that their nuclei would immediately fly apart into nucleons and nuclear fragments and never become atoms.
Traditionally, the discoverers of a new element chose its name. Then the International Union of Pure and Applied Chemistry (IUPAC) officially approves the proposed name. However, for several of the transfermium elements (Z = 102 and higher) there had been competing claims to their discovery. Recently, the names for elements have been decided up to Z = 109. Table 8-1 lists the currently approved IUPAC names.
It has been possible to study the chemical properties on the macroscopic scale for elements as massive as einsteinium (element 99) and on the tracer scale for elements as massive as seaborgium (element 106). The elements beyond the actinides in the Periodic Table are termed the "transactinides" and are shown in a Modern Periodic Table as in Fig. 8-2 with all of the undiscovered elements through number 118 in their expected places.
Element Number |
IUPAC Proposal |
Symbol |
101 |
Mendelevium |
Md |
102 |
Nobelium |
No |
103 |
Lawrencium |
Lr |
104 |
Rutherfordium |
Rf |
105 |
Dubnium |
Db |
106 |
Seaborgium |
Sg |
107 |
Bohrium |
Bh |
108 |
Hassium |
Hs |
109 |
Meitnerium |
Mt |
The yields of the most massive elements produced in bombardments of target nuclei with "heavy" ions become extremely small with increasing atomic number, dropping to as little as one atom per week of bombardment for elements as massive as atomic number 112. The half-lives decrease into the millisecond and the microsecond range so that identification of the new nuclei becomes increasingly difficult. These half-lives would be impossibly short were it not for the presence of closed shells of nucleons to increase nuclear stability.
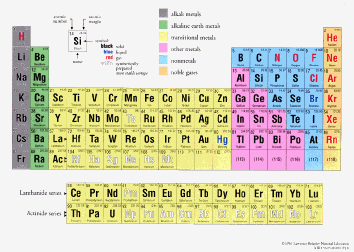
It has been possible to study the chemical properties of rutherfordium, hahnium and seaborgium using the advanced techniques of one-atom-at-a-time chemistry, with the result that these properties in the main are consistent with those expected on the basis of extrapolation from those of their lower-mass homologues in the Periodic Table, hafnium, tantalum, and tungsten. It should be possible to similarly study the chemical properties of bohrium (107) and hassium (108), the homologues of rhenium and osmium. However, the chemical properties cannot be determined reliably in detail from trends exhibited by the lighter homologues, because of the important role played by relativistic effects (the fact that some electrons are moving at velocities near the speed of light) in these more massive elements.
It will surely be possible to study the macroscopic properties of fermium (element 100) and not out of the question that this will eventually be done for mendelevium (element 101). The art of one-atom-at-a-time chemistry will advance far beyond what can be imagined today to make it possible to study the chemistry of most massive elements. All of this will result in the delineation of relativistic effects on the chemical properties of these very massive elements, which might thus be substantially different from those expected by simple extrapolation from their less massive homologues in the Periodic Table.
Table 8-2. Summary of the reactions and methods used in the discovery of the actinide and transactinide elements. See Chapter 7 for an explanation of the reaction notation.
Element
|
|
Method of Discovery
|
Year
|
neptunium (Np)
|
238U(n,b -)239Np
|
Chemical Separation
|
1940
|
plutonium (Pu)
|
238U(2H,2n)238Np
|
Chemical Separation
|
1941
|
curium (Cm)
|
239Pu(4He,n)242Cm
|
Chemical Separation
|
1944
|
americium (Am)
|
239Pu(n,g )240Pu
|
Chemical Separation
|
1945
|
berkelium (Bk)
|
241Am(4He,2n)243Bk
|
Chemical Separation
|
1949
|
californium (Cf)
|
242Cm(4He,n)245Cf
|
Chemical Separation
|
1950
|
einsteinium (Es)
|
|
Chemical Separation
|
1952
|
fermium (Fm)
|
|
Chemical Separation
|
1953
|
mendelevium (Md)
|
253Es(4He,n)256Md
|
Recoil Method, Chemical Separation
|
1955
|
nobelium (No)
|
246Cm(12C,4n)254No
|
Recoil Method, Chemical Separation
|
1958
|
lawrencium (Lr)
|
249/250/251/252Cf(10/11B,xn)258Lr3
|
Direct a Counting
|
1961
|
rutherfordium (Rf)
|
249Cf(12C,4n)257Rf
|
Parent-Daughter a Correlation
|
1969
|
dubnium (Db)
|
249Cf(15N,4n)260Db
|
Parent-Daughter a Correlation
|
1970
|
seaborgium (Sg)
|
249Cf(18O,4n)263Sg
|
Parent-Daughter-Granddaughter a Correlation
|
1974
|
bohrium (Bh)
|
209Bi(54Cr,n)262Bh
|
|
1981
|
hassium (Hs)
|
208Pb(58Fe,n)265Hs
|
|
1984
|
meitnerium (Mt)
|
209Bi(58Fe,n)266Mt
|
|
1982
|
110 (unnamed)
|
208Pb(62,64Ni,n)269,2711105,6
|
Mass Separator
|
1991
|
111 (unnamed)
|
209Bi(64Ni,n)272111
|
|
1994
|
112 (unnamed)
|
208Pb(70Zn,n)277112
|
|
1996
|
1When more than one reaction is given, it means that a sequence of reactions was necessary to discover the element.
2The Es and Fm reactions were not done in a laboratory setting; multiple neutron captures, followed by successive beta decays were the result of a thermonuclear explosion.
3A mixture of four Cf isotopes were bombarded simultaneously with a beam containing 10B and 11B. The xn means that different numbers of neutrons were emitted, depending on the actual combination of target and beam used to produce Lr.
4A velocity separator is used to separate reaction products based on the fact that reaction products of different masses will be emitted with different velocities.
5Three different claims to element 110 have been made by teams from Lawrence Berkeley National Laboratory, the GSI Laboratory in Germany and a collaboration from Dubna in Russia and Lawrence Livermore National Laboratory. As of now, no one claim has been given sole credit for the discovery.
6At GSI two different reactions were used to produce two different isotopes of element 110.
Chapter Contents
|
|