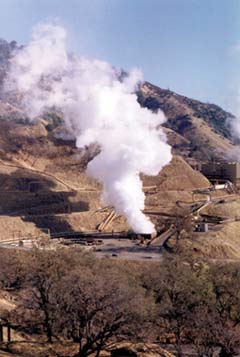 |
Berkeley Lab scientists are working to understand the history and characteristics of The Geysers in Northern California, the largest geothermal electricity producer in the world, which is showing effects of overexploitation. |
Landing in Hot Water
"I'm a hydrogeologist by training," says Marcelo Lippmann of the Earth Sciences Division's Energy Resources Department. "If you add heat to that, you get a geothermal-reservoir engineer."
Lippmann heads ESD's Geothermal Energy Development Program, which got its start in the wake of the oil crises of the 1970s. In those years the federal government was eager to investigate any sources of energy that might offset the need for expensive foreign oil, especially clean, renewable ways of generating electricity.
A quarter of a century later energy economics are different, and approaches to geothermal energy are more sophisticated. Geothermal heat pumps have been developed that make use of the normal temperature of the ground a few feet below the surface, a superbly efficient heat-exchange technology for buildings. Cities as different as Klamath Falls, Oregon, and Paris, France, heat apartments, homes, factories, and greenhouses, and even keep streets free of snow, using circulating water heated by hot rock or extracted from geothermal wells.
But electricity from turbines powered by rock-heated steam or hot water is still the most valued form of geo-thermal energy, and the most valuable resources of all are "dry steam" reservoirs like Northern California's Geysers, where hot rock several kilometers below the surface vaporizes "ancient" waters, producing live steam under pressure. In systems like these, geothermal-reservoir engineering is essential.
"They built the first 12-megawatt power plant at The Geysers in 1960," says Lippmann. "Now there are over 600 wells and 20 plants providing over a thousand megawatts of powe-the largest geothermal electricity producer in the world. But steam production peaked in 1988 and has been declining ever since."
Like a water well, The Geysers reservoir has started to run dry from use. To maintain steam pressure, water is being pumped back into the reservoir. Unlike water that has slowly trickled deep into the ground and has had time to heat up, however, the injected water is cold and may gradually cool the hot rock itself. How much cold water can be returned to the reservoir without damping the furnace?
"We're using several approaches to understand the history and present characteristics of The Geysers reservoir, which will help us predict performance there," says Lippmann. "For example, we use an array of digital seismometers to measure the micro-earthquakes that occur when steam is extracted or water is injected."
By using seismic data from natural and artificial events to build up 3-D images, and by seeking to understand how microearthquakes are related to underground geologic structures and reservoir properties, geothermal program researchers-including Ernest Majer of the Geophysics and Geomechanics Department, with Ann Kirkpatrick and John Petersen at The Geysers and Thomas Daley and Mark Feighner at the Rye Patch reservoir in Nevada-hope to learn where and where not to drill, where and where not to inject water, and what other factors may help sustain reservoir productivity far into the future.
Donald DePaolo and Mack Kennedy use isotope geochemistry to determine the sources of fluids and gases at The Geysers and other geothermal reservoirs around the world, including regions in the Philippines and Tibet. The ratio of common helium-four to rare helium-three, produced by radioactive decay deep in the mantle, can reveal the proportion of ground water to magmatic fluid, or of injected water to these "indigenous" fluids.
Ki Ha Lee creates images of geothermal reservoirs by measuring the varying electrical resistivity of underground structures such as fractured rock. Peter Persoff studies the properties of veined and fractured rock in the laboratory, hoping to understand how the channels essential to water circulation open and close over time.
These methods and more contribute to computer programs for simulating the behavior of existing reservoirs and evaluating new ones. The family of tough programs (Transport of Unsaturated Groundwater and Heat), developed by Karsten Pruess in the 1980s and extended by many other researchers including Stefan Finsterle, George Moridis, Curtis Oldenburg, and Yu-Shu Wu, has become a powerful tool for projecting the behavior of water, vapor, and heat in geothermal reservoirs-not to mention hydrocarbons, solvents, toxic waste, and practically anything else that flows underground.
The Pacific Ring of Fire, the mid-ocean ridges and continental rift zones, and a great many anomalous hot spots around the globe represent a vast worldwide potential for geothermal energy development. But in the short term, says Lippmann, geothermal's future lies
in direct uses-hot water heating such as that in Klamath Falls-and in isolated areas without access to cheap electricity. As long as fossil fuels are cheap, electricity from geothermal sources will seem expensive.
"On the other hand, fossil fuels will never be as clean as geothermal energy," Lippmann says. "If our political leaders accept global warming as a real phenomenon and decide to get serious about clean energy, geothermal sources could provide as much as ten percent of this country's electrical energy."
Ice On fire
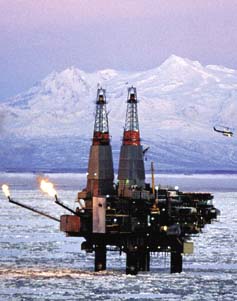 |
Mysterious fizzing and bubbling during deep-sea drilling in the 1970s was a clue to the existence of methane hydrates.
|
|
 |
In 1964, oil workers in Siberia struck what they called "frozen natural gas;" some of the Soviet roughnecks amused themselves scooping up handfuls of slush, striking a light, and throwing flaming snowballs at their comrades.
In fact the Soviets had discovered huge deposits of methane hydrate, methane gas molecules trapped inside cages of water ice in a form so concentrated that, when melted, the gas expands to 164 times its frozen volume.
Gas hydrates are plentiful in permafrost; in the 1980s enormous quantities were found on Alaska's North Slope. Hydrates are even more plentiful under the sea. During deep-sea drilling in the 1970s, they were found to be the source of mysterious fizzing and bubbling-although an intact core sample of sea-bottom methane hydrate wasn't recovered until 1981.
U.S. deposits of hydrates equal many thousand times the nation's annual consumption of natural gas. So much gas is locked up in hydrates worldwide, in fact, that one estimate, described as "conservative" by the U.S. Geological Survey (USGS), puts the amount of carbon in hydrates at twice the amount of carbon in all known fossil fuels on Earth.
There are profound reasons for approaching methane hydrates cautiously. Methane is a potent greenhouse gas, far more effective than carbon dioxide at trapping heat in the atmosphere. During the ice ages, catastrophic releases of methane from sea-floor hydrates may have triggered landslides on the continental shelves, then bubbled into the atmosphere, contributing to wildly oscillating global temperatures.
"As yet we have no idea if this is a commercially viable resource," says George Moridis of ESD's Department of Hydrogeology and Reservoir Dynamics. "It may be like gold in
seawater-there's a lot there, but nobody knows how to extract it economically."
This fall, in order to establish a baseline for estimating the commercial potential of gas hydrates, Moridis, working with researchers such as Larry Myer, head of ESD's Energy Resources Department, and Timothy Collett of USGS, will apply a sophisticated numerical model to characterize deposits and assess production in wells at Milne Point, Alaska, near Prudhoe Bay.
"We'll have a thermodynamically correct 3-D model of the reservoir, with a minimum of simplifying assumptions. We'll soon find out if any of those assumptions are wrong, or if there are circumstances we failed to take into account." The Alaska site has an important advantage, Moridis says. "Unlike Siberia, the natural gas deposits associated with the hydrates are limited, and the production wells will be horizontal and drilled completely in the hydrate zone, so we'll know the gas we get is coming from the hydrates and nothing else. It's the first clear-cut case."
One challenge, he says, is that "in nature, the formation of hydrates is a very strange process. How do some molecules serve as nucleation points for hydration? What part does pressure play? Clays are often present, but their role is unknown. As yet there is no reliable way to make gas hydrates predictably in the laboratory."
The source of the methane is itself a mystery. Some of it comes from the same source as swamp gas, the breakdown of organic material by microorganisms. Some comes from deep inside the Earth; is it also biogenic? If so, what kind of microorganisms are responsible?
Getting the gas out of the ice presents a different set of challenges. The main techniques involve lowering the pressure or increasing the temperature, sometimes adding agents such as alcohol or salt water to alter the pressure/temperature equation. "Depressurization seems most effective," says Moridis, "although in Alaska we have deep, high temperature brine aquifers, so we can exploit both the thermal stimulation and the inhibiting effects of the salt water."
The porosity of the deposits is as yet unknown; gas can't move unless there is enough porosity in the hydrates, "but if there is enough porosity, we are confident of high production," Moridis says. Because the Milne Point deposits are shallow, they will be drilled "horizontally," on a sloping path that covers more lateral area than a vertical borehole.
Gas hydrates have yet to establish their worth-but they have already established themselves as a nuisance to oil and gas producers on land and undersea by contributing to clogged pipelines, well-head blowouts, and well-casing failures. Sensitive to pressure and temperature, they can readily change state while being drilled; where ice suddenly changes to gas, the ground can collapse and even bring down drill rigs. Part of the answer to dependable production lies in preventing such unpleasant surprises.
Like geothermal energy, methane hydrates are an alternative energy source for which there is no immediate economic pressure. "As long as oil and gas are cheap, why should energy companies invest the time and money?" Moridis asks. "But someday the picture will change, and when it does, we hope to have solid answers to the question of methane hydrate's potential as an energy source."
Squeezing the Glass Sponge
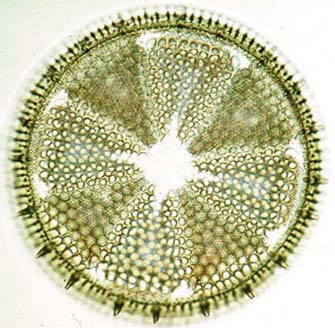 |
Diatomite is porous but impermeable rock made up of fossilized skeletons like the one pictured above, the remains of innumerable microscopic plants that once lived in sunny waters. |
Since the last age of the dinosaurs, the silica remains of countless single-celled plants have settled on the floors of freshwater lakes and shallow seas, building up thick deposits of diatomite-rock made of microscopic hollow glass skeletons.
In sixth-century Constantinople, Byzantine architects built the wide, soaring dome of Agia Sophia with blocks of lightweight diatomite; in the 1860s, Alfred Nobel created dynamite by saturating the absorbent stuff with nitroglycerin.
"Dry diatomite will float on water; its porosity is twice that of sand," says Tadeusz Patzek of ESD's Department of Hydrogeology and Reservoir Dynamics, an associate professor of Petroleum Engineering at UC Berkeley. "At up to 60 percent empty space, it's a natural sponge for crude oil."
The problem with a glass sponge is that it is impermeable. Diatomite and other low-permeability rocks contain a third of the world's reserves of crude oil-the vast diatomite beds near Bakersfield are a thousand feet thick and hold as much as three billion barrels of oil-but most of it is still in the ground.
"The only way to get to the oil is to fracture the rock," Patzek explains. "In the South Belridge field west of Bakersfield, the oil companies started by drilling production and injection wells 250 feet apart. These wells have massive vertical fractures, hundreds of feet tall and hundreds of feet long, at times radiating outward in every direction. But then the companies began drilling wells closer together, 180 feet apart, and now they're going to 80 feet apart. The fractures start to interact and cause problems" -problems such as the ground sinking 20 feet in places, "crevices that can swallow a truck," production wells that bring up too much water with the oil, or boreholes flattened like soda straws.
Patzek, working with Larry Myer, Ernest Majer, and representatives from the oil companies and other Department of Energy national laboratories, has studied ways to improve oil recovery in the South Belridge field, with results applicable to other "tight" reservoirs.
Waterflooding, the most common technique, is done by injecting water at very high pressure. It serves a double purpose, splitting the rock and filling the voids when oil is removed, which supports and maintains pressure in the reservoir. Carbon dioxide and steam perform similar functions; steam is a good injectant because it does not come in direct contact with the oil-instead it drives oil from the rock by heating-but it can be expensive and hard to control.
"You can't produce from this kind of rock without injecting something, but the injection must be controlled," says Patzek, "otherwise fractures grow unpredictably and irregularly, sometimes branching out and joining. Control is the essence of our work."
Patzek and his colleague Dmitriy Silin have devised a "smart" controller for water, steam, or carbon dioxide injection. As the fluid is injected, a computer gathers data from the injection well and from remote sensors, monitoring the well's current status and comparing it to the history of the well and the whole field. The information is used to control a valve that continuously varies injection rate and pressure.
"When the pressure drops and the rate of fluid flow increases, it's because the fracture is growing," says Patzek. "Existing controllers try to maintain constant pressure; during accidental fracture extensions, this can make the fracture run away. Our smart controller balances all the variables and remains stable even during catastrophic extension."
The smart controller measures a well's injection rate and pressure as often as once every second. Other data may come from high-pressure pulses sent down the borehole to create seismic signals. Overall oil and water production data from the entire field ensure that injection wells are operated as part of the total reservoir system.
In the near future Patzek hopes to add a new source of field-wide data in the form of inexpensive solar-powered tiltmeters that continuously monitor ground movement and radio the data to the controllers.
"Because fracturing and fluid replacement are essential to productivity, but also interdependent, we have to macromanage the whole field," says Patzek. "Optimal supervision will employ arrays of smart controllers at all injection wells, taking data from sensors throughout the field and working with a computer model of the whole reservoir."
By devising ways of extracting more of the oil stored in low-permeability rocks, Patzek and his colleagues are hedging bets against the future. "In some ways we have become too efficient," he says, "to the point where we are overproducing worldwide. Because the price of crude is so low, right now companies are avoiding major investment in sophisticated technologies. One thing we can say for sure-sooner or later, that situation will change."
Rocks heated in the formation of the solar system . . . hydrated gas of mysterious origin . . . the oily remnants of living creatures, locked in the glassy remains of other creatures . . . In the decades and centuries to come, we will need all the strange and various forms of energy that Earth has stored within itself, and more. end
|