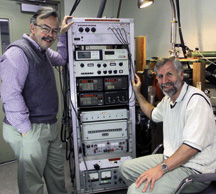 |
When Eugene Haller and Wladyslaw Walukiewicz (pictured) and their colleague Oscar Dubon squeezed copper-doped germanium, its conductivity increased a trillion-fold. |
|
 |
Germanium, doped with copper and cooled to the temperature of liquid helium, is ordinarily a good insulator. But it becomes an astonishingly good conductor of electricity when squeezed along one axis of its crystalline structure. The surprising discovery was made by Oscar Dubon, Eugene Haller, and Wladyslaw Walukiewicz of the Materials Sciences Division.
"In my mind, the great thing is shown in this graph," Haller says, pointing to a plot that displays the resistivity of a copper-doped germanium sample abruptly dropping as pressure increases. "A little pressure induces a change of over a dozen orders of magnitude-one trillion fold!-in conductivity."
The increase in conductivity is so rapid and so large that the effect may be useful in designing instruments to detect extremely small changes of temperature or pressure. Even more important, the phenomenon offers condensed-matter researchers a new way to study the insulator-metal transition.
The overlapping electron orbitals of an undoped semiconductor form distinct energy bands, with lower-energy valence bands completely full of electrons and higher-energy conduction bands empty. If there are no extra charge carriers in these bands, the material is an insulator. But if the bands lie relatively close together on the energy scale, even a little heat can give valence-band electrons enough energy to leap to the conduction band. This makes the crystal a metal-like conductor.
The transition effect can be enhanced or modified by doping. When some of the pure crystal's atoms are replaced by others such as phosphorus, electrons are added to the conducting band. Doping with boron leaves positively charged "holes" in the valence band, another way to allow charge to flow.
Copper can contribute holes to germanium's valence band, but if concentrations are low, the copper atoms are widely separated, and charge can move only by "hopping" between copper sites-an unlikely process. The germanium still acts as an insulator.
"We decided to take germanium that was doped with copper but was still a good insulator, and apply uniaxial stress," Walukiewicz explains. By simply squeezing it in a vise, the researchers applied over four kilobars of pressure to a one-by-one-by-five millimeter chip of copper-doped germanium.
They found that pressure applied along one direction changed the electron orbitals around the copper atoms, effectively enlarging these orbitals enough so that even at low concentrations they overlapped and permitted conduction.
Moreover, the overlapping orbitals of the copper atoms now form their own separate band, with properties that depend on charge and electron spin. Solid-state researchers have been intrigued by these doping-induced bands since they were first proposed by theorist John Hubbard 40 years ago.
Hubbard bands are exceedingly difficult to study, however, because in most semiconductors the higher-energy Hubbard component merges with the crystal's valence band near the insulator-metal transition point. Uniaxially stressed copper-doped germanium doesn't have this problem. It represents a pristine case of isolated Hubbard bands.
Not only have the researchers seen a huge physical effect, an increase in conductivity of many orders of magnitude achieved simply by applying pressure, says Haller, "but it is intrinsically interesting to be able to study the Hubbard band in a new state, clearly separated from the valence band of germanium. We can do that for the first time."
New Fluorescent Probes for Biological Materials
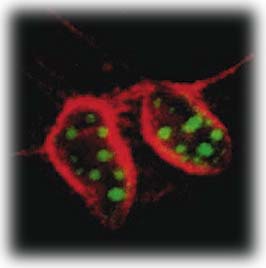 |
In this cross-section of mouse cells labeled with two different sizes of semiconductor nanocrystals, nuclei show up as green and actin fibers as red under the same illumination. |
|
 |
Some of the more shadowy secrets of biology may soon be illuminated through the use of a new type of fluorescent probe developed by scientists at Berkeley Lab and the University of California at Berkeley (UCB).
A joint Berkeley Lab-UCB research team led by Paul Alivisatos and Shimon Weiss has announced the development of nanometer-sized crystals of semiconductors, such as cadmium selenide and cadmium sulfide, that can be used as fluorescent probes for the study of biological materials.
These semiconductor nanocrystals offer a distinct advantage over conventional dye-molecules in that they emit multiple colors of light, which means they can be used to label and measure several biological markers simultaneously. The unique optical properties of these semiconductor nanocrystals also hint at the possibility of observing changes that take place in labeled biological systems, such as living cells, over a period of time. The research was reported in the September 25, 1998 issue of Science.
Alivisatos is a leader in the production by chemical means of semiconductor nanocrystals, simple inorganic solids consisting of a hundred to a hundred thousand atoms. He holds a joint appointment as a UCB professor of chemistry and a senior staff scientist with Berkeley Lab's Materials Sciences Division (MSD). Weiss, a staff scientist with MSD, is an authority on single molecule fluorescence and spectroscopy. He approached Alivisatos with the proposal for this joint study.
Other members of the team who co-authored the paper in Science were Marcel Bruchez Jr., who also holds a joint Berkeley Lab-UCB appointment, and Mario Moronne and Peter Gin, with the Lab's Life Sciences Division.
"Form follows function" is the golden rule in cell biology, which is why microscopy has been the heart and soul of this research field, and fluorescent-labeling one of its most widely used tools. In fluorescent labeling, markers, usually antibodies that attach themselves to specific proteins, are tagged with dye-molecules that fluoresce or emit a specific color of light when stimulated by laser light, usually from a confocal microscope.
"Sometimes, in order to fully characterize a sample-a population of cells, for example-you need to look at combinations of markers," says Alivisatos. Such measurements require multiple-color light emissions which are difficult to obtain with conventional dye molecules.
"Ideal probes for multicolor experiments should emit at spectrally resolvable energies, should have a narrow, symmetric emission spectrum, and the whole family should be excitable at a single wavelength," the authors of the Science paper wrote.
Semiconductor nanocrystals met these demands in a "dual emission from single excitation" labeling experiment on mouse tissue cells called 3T3 fibroblasts. A core nanocrystal of cadmium selenide was enclosed within a shell of cadmium sulfide to boost the amount of fluorescence and reduce photochemical degradation. This core-shell combo was then enclosed within a shell of silica for water solubility and biocompatibility.
With earlier work by Alivisatos having shown that the color of light emitted by a semiconductor nanocrystal depends upon its size, the mouse cells were labeled with two different sizes of core-shell nanocrystals. It was also known that modifying the surface of the silica shell can be used to selectively control its attachment to components within a cell. In this case, the smaller nanocrystals (two nanometers), which fluoresced green, were modified to penetrate the nucleus of each cell, and the larger nanocrystals (four nanometers), which emitted red light, were modified so that they would attach themselves to actin filaments along the outer cell membrane.
Using wide-field microscopy, the green and red labels were clearly visible to the naked eye and could be photographed in true color with an ordinary camera. Confocal microscopy images showed that cell nuclei had been penetrated with the green probes and the actin fibers had been stained red. After repeated scans, the nanocrystal labels showed far less photobleaching than would have occurred in the control sample labeled with conventional dye molecules.
"The development of semiconductor nanocrystals for biological labeling gives biologists an entire new class of fluorescent probes for which no small organic molecule equivalent exists," the authors of the Science paper wrote. "These nanocrystal probes can be complementary, and in some cases may be superior to existing fluorophores."
Weiss and Alivisatos and their co-authors also believe that semiconductor nanocrystals could be applied to x-ray- and electron-based imaging techniques, and could serve as tunable infrared dyes for detecting fluorescence in, among other things, blood samples.
Hot Sparks from Metallic Glass
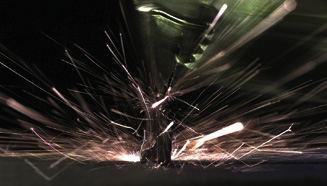 |
When a notched sample of zirconium-based metallic glass is struck by a pendulum in air, it fractures and throws off burning sparks with a temperature of 3,175 degrees Kelvin. |
|
 |
A team of researchers in the Materials Sciences Division, led by Robert Ritchie, have found that a new kind of zirconium-based metallic glass has a startling property: when fractured in air, it throws off showers of bright, hot sparks.
The alloy of zirconium, beryllium, and three other metals melted together was discovered at the California Institute of Technology by William Johnson and Atakan Peker; it is one of the first metallic glasses that can be made in bulk and formed into strong, hard, useful objects.
"We were measuring how much energy it took to fracture the material when we stumbled onto the light emission," says Christopher Gilbert, a postdoctoral fellow in Ritchie's High Performance Metals Program.
The researchers mounted notched specimens of the metallic glass in a pendulum impact device. In air, when struck by the pendulum, the specimens snapped and sent out bright sparks whose color indicated a temperature of 3,175 degrees Kelvin. Under a microscope, blobs of melted material were seen on the fracture surface, meaning local temperatures must have exceeded 993 K, the temperature at which the metallic glass liquefies.
The sparks in air were caused by burning particles thrown off from the fracture surface. The same experiment in a nitrogen atmosphere produced no visible sparks, but emission was detected in the infrared at 1,400 K.
"Zirconium and titanium will burn in air, if you get them hot enough," says Ritchie, "but the real question is the temperature we observe in nitrogen-1,400 K in the absence of intense oxidation and pyrophoric activity."
Team member Joel Ager surmises that the temperature rises rapidly as the material is deformed "partly because metallic glass has terrible conductivity for a metal. It can't get rid of the heat. But this can't be the whole story."
Unlike pure metals and most metal alloys, metallic glasses have no regular crystalline structure. This lack of long range order is related to such desirable features as strength and low damping-the ability to deliver a really big bounce-which is one reason why the premier use for zirconium-based metallic glass is the manufacture of expensive golf club heads. The golf clubs made by inventor Johnson's company, Amorphous Technologies International, have unusual springiness, a "soft" feel, and an almost ideal density between that of stainless steel and titanium, currently the connoisseur's choice.
Only recently has it been possible to obtain metallic glass in enough bulk to make even a golf club head. In the past, to prevent segregation and crystallization of the melt required such rapid cooling-in a thousandth of a second, at a rate of about a million degrees Celsius per second-that only very thin wires and ribbons could be cast.
Zirconium-based metallic glasses can be cooled much more slowly, at about 10 degrees C per second; they achieve their glassy, disordered state by alloying metals with dramatically different atomic sizes and chemical characteristics.
Even slow-cooling metallic glass doesn't cool slowly enough for castings larger than about four inches. And team member Valeska Schroeder has shown that despite the amorphous nature of the zirconium-based glass, it is no more resistant to corrosion than its crystalline components. While there is much yet to learn, the many unusual properties of bulk metallic glasses hold promise for important applications.
As for unexpected light emissions from the zirconium-beryllium alloy, Ritchie says, "These extremely high temperatures aren't Polywater, not a delusion-they're real. And they demand explanation."
|