The recent announcement of molecular-sized computer switches
has renewed predictions of hand-held supercomputers in the near future. Practical benefits
from dramatically reducing the size of data processors, however, will only be realized if
there is an equally dramatic reduction in the size of data storage devices. A
significant step towards downsizing magnetic storage disks has been achieved by
researchers working at Berkeley Lab's Advanced Light Source (ALS). They are producing the
first high quality images of "quantum wells" an energy state in which an
electron is sandwiched between two layers of atoms so that its motion is confined to a
single dimension.
"As magnetic storage devices become more densely packed with information, reading
the data becomes more difficult," says Zi Qiang Qiu, a solid state physicist who
holds a joint appointment with the Lab's Materials Sciences Division (MSD) and the Physics
Department of the University of California at Berkeley. "A better understanding of
the physics behind quantum well states should enable us to make much more sensitive
reading heads for magnetic disks, which in turn, should make it possible to increase disk
storage density."
Today's typical magnetic storage device is a plastic disk coated with multiple layers
of metallic thin films. Data is stored by converting electrical signals representing the
0s and 1s of the binary code into magnetized areas, called "bits," on the disk's
metallic film. Orienting a magnetic bit in one direction represents a 0 and in the
opposite direction a 1. The smaller the bits that can be written and read, the more
densely a disk can be packed with data.
If all magnetic devices could be pushed into the wavelength limit of an electron moving
through a solid -- the nanometer scale -- storage capacity would be significantly raised
above that of today's record best. The key is to confine the movement of the electron.
"In our experiments, the movement of the electron is restricted so that it bounces
back and forth at an interface, perpendicular to the surfaces, to form a standing
wave," says Qiu.
Images obtained at the ALS by Qiu and his colleagues revealed that the amplitude of a
confined electron wave is modulated by a mathematical function which surrounds the
electron wave like a longer wavelength "envelope." This envelope surrounding and
modulating the electron wave determines the energy states of the quantum well. As the
electron's wavelength gets shorter, quantum well energies increase and vice-versa.
Quantum well energy states are believed to be the basis for "oscillatory
coupling" and giant magnetic resistance (GMR). Oscillatory coupling relates magnetic
orientations in multiple layers of metallic films to film thickness. GMR is the phenomenon
whereby exposure to a small magnetic field induces a large change in electrical
resistance. Both effects could be crucial to boosting disk storage density.
Manufacturers of magnetic storage devices are already beginning to exploit these
effects through structures in which two magnetic layers are separated by a metallic but
nonmagnetic spacer layer. The technology, however, is in its infancy and there is much
room for developmental improvement. The wave function data being gathered at Berkeley Lab
is considered vital to successful development.
For their studies, the Berkeley researchers varied the thickness of their samples by
making them wedge-shaped. This technique enabled the scientists to simultaneously vary the
thickness of their metallic layers, creating nano-sized electron interferometers for
observing quantum well states in each layer.
In one study, they deposited a wedge-shaped layer of magnetized cobalt over a nickel
substrate then covered it at right angles with a spacer wedge made of copper. The
variation in photemission intensity from a single scan across the sample with ALS light
was the result of wave interferences from quantum well states in the two layers.
In another study, a layer of nickel only one atom thick was embedded between two wedges
of copper. This enabled the Berkeley scientists to map the amplitude of their wave
functions in much the same way that vibrations of a string can be analyzed by lightly
touching it at different spots.
"Touching the string at an antinode or at a node causes considerably different
effects in the vibration," says Qiu. "By systemically changing the contact
position along the string, it is possible to map out the spatial variation of the
vibration amplitude. The nickel layer is analogous to the touch of a finger, causing the
vibrational modes of our wave functions to respond accordingly."
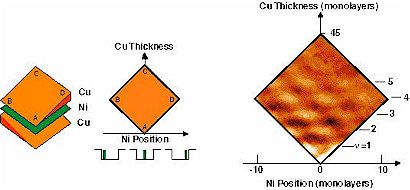
SANDWICHING A SHEET OF NICKEL ONE-ATOM THICK IN BETWEEN WEDGE-SHAPED SAMPLES OF COPPER
CREATES TINY ELECTRON INTERFEROMETERS FOR OBSERVING QUANTUM WELL STATES (AS SEEN AT RIGHT)
IN EACH COPPER WEDGE
Critical to the success of Qiu and his colleagues in this work has been the quality of
the x-ray beams generated at the ALS. One of the first of the "third-generation"
synchrotron light sources, the ALS produces the world's brightest beams of ultraviolet
light and low-energy x-rays. These beams are ideal for the study of surfaces and
interfaces on a nanometer scale.
Qiu and his colleagues worked at an undulator beamline called "The
Spectromicroscopy Facility." The beam at this facility delivers a trillion photons
per second, focused down to a spot size of 50 to 100 microns (about the diameter of a
human hair). This tiny spot size makes the detection of quantum interference effects
possible.
"We now have sufficient understanding of quantum well states to begin learning
more about how these states are coupled with magnetic properties," Qiu says.
"Our aim is to reach a level of theoretical understanding and experimental control
that will make the wave function engineering of magnetic materials comparable to the
bandgap engineering of semiconductors."
Collaborating with Qiu on this project are Roland Kawakami, Hyuk Choi, Ernesto
Escorcia-Aparicio, Maria Bowen, Jason Wolfe, Elke Arenholz, and Z.D. Zhang from the UC
Berkeley Physics Department, plus Eli Rotenberg, who oversees use of The Spectromicroscopy
Facility for the ALS, and Neville Smith, the deputy for the ALS scientific programs.
Discussions of their work have already appeared in Science, Nature, and other
scientific journals. |