BERKELEY, CA — Joseph Orenstein, a staff scientist
in Berkeley Lab's Materials Sciences Division and a professor of physics
at the University of California at Berkeley, applied a technique called
"time-domain terahertz spectroscopy" to study the electronic
properties of the high-Tc superconductor BSCCO. When he and his colleagues
found peculiar -- and at first unbelievable -- results, their discoveries
encouraged Séamus Davis to search for evidence of granular superconductivity
in the same material.
The superconducting state
Electrons are fermions, particles that cannot occupy the same quantum
state in a system. But when bound together as "Cooper pairs,"
the pairs are bosons, which can occupy precisely the same quantum state
-- all at the same time. Thus electron pairs can form a "condensate"
that gives rise to all the spectacular electromagnetic effects displayed
by superconductors, including the absence of electrical resistance.
 |
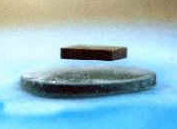 |
|
A spectacular electromagnetic
effect of superconductors: a superconductor can levitate a magnet,
because after making the transition from the normal to the superconducting
state, the superconductor excludes magnetic fields from its interior.
|
|
Not every electron finds a partner, however. Unpaired electrons are described
as quasiparticles, whose properties include finite lifetimes.
"The standard picture of superconductor electrodynamics is the two-fluid
model," says Orenstein, "in which the medium behaves as a superconducting
fluid -- a superfluid -- of Cooper pairs, which is permeated by
a normal fluid consisting of quasiparticles."
Electrical charge is carried by electrons, but also by the oppositely-charged
absences of electrons called holes. Thus Cooper pairs can also be made
of holes. Quasiparticles too may be particle-like or hole-like.
Quasiparticles are difficult to study in a superconductor because their
properties are usually hidden by the superfluid with which they coexist.
The resistance of the normal fluid is undetectable, for example, because
it is "shorted out" by the resistanceless condensate. So Orenstein
set out to study quasiparticle properties by measuring conductivity using
alternating rather than direct current.
When direct current flows through a superconductor, there is no resistance,
no voltage, and the electric field strength is exactly zero. While direct
current does induce a non-zero magnetic field, it is measurable only near
the surface, to a depth known as the penetration depth.
Because the condensate has mass, however, alternating current requires
force to repeatedly accelerate and decelerate the charge carriers. Thus
there is a measurable electric field and voltage, although no resistance
(because current and voltage vary out of phase).
Like a magnetic field, the ac electric field is measurable only to the
penetration depth, below which it is "screened." The strength
of the screening and the shallowness of the penetration depth vary with
the density of the superfluid.
When normal fluid is present, it also contributes to the current. Just
as in a normal metal, this current encounters resistance. Energy is converted
to heat and dissipates at a rate that peaks when the ac frequency exactly
matches the scattering rate of the quasiparticles that make up the normal
fluid.
The higher the scattering rate, the shorter the quasiparticle lifetime,
an important parameter of various high-Tc superconductors.
The puzzle of quasiparticle lifetimes
When Orenstein began his ac investigations, measurements of quasiparticle
lifetime in BSCCO and in a very similar material, YBCO (yttrium barium
copper oxide), had yielded quite dissimilar results. Teams who had measured
YBCO with microwave frequencies found that quasiparticle lifetimes rapidly
increase below the superconducting transition temperature, to more than
100 times that in the normal state.
Microwave measurements of BSCCO, on the other hand, failed to observe
a dissipation peak, which is needed to pin down quasiparticle lifetimes.
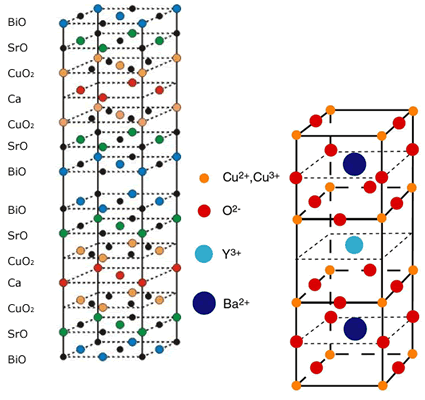 |
|
The high-temperature superconductors
BSCCO and YBCO are similar in many respects, including their Perovskite
structure, but their electronic properties reveal suprising differences.
|
|
At Berkeley Lab's Advanced Light Source, a team headed by Z. X. Shen
and Zahid Hussain used a technique called ARPES (angle-resolved photoemission
spectroscopy) to measure quasiparticle lifetime in BSCCO; ARPES looks
at the electrons emitted from the surface of a material when it is illuminated
with high-frequency radiation. Surprisingly, their ARPES measurements
suggested that in BSCCO, unlike YBCO, quasiparticle lifetime increased
only by a factor of three below the transition temperature.
Were the results different because the materials were different, or because
the measurement techniques were incompatible?
"One of the frustrating things about high-Tc superconductors is
that we have many experimental probes for investigating them, but each
works best on only one or two members of the cuprate family," Orenstein
remarks.
Finding the right frequency
It seemed likely that the missing dissipation peak in BSCCO lay at frequencies
above the range of typical microwave spectroscopy techniques. Orenstein
turned to time-domain terahertz spectroscopy, with frequencies between
microwaves and the far infrared. With the terahertz spectrometer he could
send a pulsed beam of trillion-cycles-per-second radiation all the way
through a slice of BSCCO only 100 billionths of a meter thick.
The terahertz pulse is produced by a short strip of silicon under voltage.
When stimulated by optical laser pulses a trillionth of a second long,
the strip responds with electric-current pulses that radiate trillion-hertz
electromagnetic waves. (In 1888, Heinrich Hertz used a similar system
of bigger conductors to produce the first radio waves.)
The terahertz waves are focused on the sample, in which they set up an
alternating current of the same frequency. The shape of this wave is altered
as it passes through the sample; on the far side, it is detected by a
reversed arrangement of focusing elements and a second silicon strip.
In studying superconductors, the terahertz spectrometer's ability to
measure the absolute phase of the transmitted pulse is crucial. Conventional
detectors read only the intensity of the wave, but Orenstein's spectrometer
records its amplitude and phase as well -- all the details of the entire
waveform.
"Remember that the quasiparticles dissipate energy, and the superfluid
screens," Orenstein says. "The signature of the condensate appears
as an out-of-phase component in the waveform, but because quasiparticles
respond in phase with the terahertz radiation, they leave a distinct signature
in the altered waveform."
Because the relative contributions of the two components to the material's
conductivity could be assessed independently, terahertz spectroscopy seemed
the ideal technique for seeking BSCCO's quasiparticle dissipation peak.
The improbable truth
The terahertz measurements produced a startling result: the two-fluid
model itself seemed to fail.
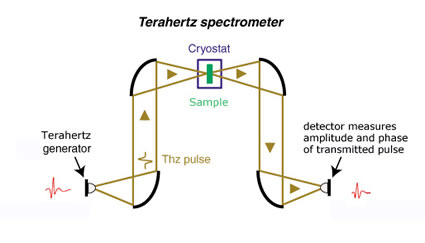 |
|
Terahertz electromagnetic pulses set
up alternating current in a thin sample; the shape of the wave is
altered as it passes through the sample, conveying information about
electronic states. |
|
"The two-fluid model suggests that when you cool a superconductor
down, you expect the normal charge carriers to convert to superconducting
pairs. The dissipative component should go down, and the screening component
should go up," Orenstein says. In BSCCO, both increased. "When
we added up the dissipation below the superconducting transition temperature,
we found some 30 percent of the total unaccounted for by the normal fluid."
Orenstein says that "eventually, we could come up with only one
explanation for this anomaly. The extra dissipation could only arise if
the superfluid density was not uniform throughout the sample. Variations
from place to place had to be almost 50 percent of the average superfluid
density! It was an extreme idea, and I never thought anybody would believe
it. I spent most of a summer worrying about it."
Learning of the Orenstein group's conclusions, Séamus Davis, using
scanning tunneling microscopy, set out to explore the possibility that
there were actual spatial variations in the conductive properties of BSCCO's
superconducting layers.
"Essentially, Séamus looks at electronic properties on the
top copper-oxygen layer of BSCCO," Orenstein explains. "He measures
energy gaps, which we believe are proportional to the density of the condensate.
His images and our measurements, which look at some 40 to 60 layers, are
in good quantitative agreement."
The story is far from over, but so far, says Orenstein, "It's been
fun. We had this prediction from looking at clues, like Sherlock Holmes.
And then Séamus goes out and actually sees the culprit."
Additional information:
|